Passive And Active Immunity Pdf Printer
The spec sheets are spot on. If you're *really* worried about things, assume a variance of 10% either way (becuase manufacturing tolerances). As for active / passive, it depends on the device. Understand how immunity works, including adaptive and innate, natural and artificial, and active and passive immunity. An Introduction to Active Immunity and Passive Immunity Search the site GO. The main difference between active and passive immunity is that active immunity is developed by the production of antibodies by person. The Immune System (pdf). Naturally acquired active immunity occurs when the person is exposed to a live pathogen, develops the disease, and becomes immune as a result of the primary immune response. Artificially acquired active immunity can be induced by a vaccine, a substance. Difference between Active and Passive Immunity in Active vs Passive Immunity, Active and Passive Immunity Immunity is defined as the body’s ability to destroy pathogens or other foreign materials and to prevent further cases of certain infecti. T1 INNATE AND ACQUIRED IMMUNITY 9 9-3 INNATE IMMUNITY The healthy individual is protected from potentially harmful micro-organisms in the environment by a. Antibodies, through passive or active immunization, play a central role in prophylaxis against many infectious agents. While neutralization is a primary function of antibodies in protection against most viruses, the relative contribution of Fc-dependent and complement-dependent antiviral activities.
Summary of recent advances
Antibodies, through passive or active immunization, play a central role in prophylaxis against many infectious agents. While neutralization is a primary function of antibodies in protection against most viruses, the relative contribution of Fc-dependent and complement-dependent antiviral activities of antibodies was found to vary between different viruses in recent studies. The multiple hit model explains how antibodies neutralize viruses and recent data on the stoichiometry of antibody neutralization suggest that the organization of viral surface proteins on viruses, in addition to virus size, influences the level of antibody occupancy required for neutralization. These new findings will improve our strategies in therapeutic antibody engineering and rational vaccine design.
Introduction
Video player untuk hp java. Traditionally, active and passive immunizations have largely been developed by trial and error. The precise mechanisms of how these immunizations actually work are often not of the highest priority, when compared to efficacy, manufacturing and safety. For example, the mechanisms of protection offered by smallpox vaccines, since Edward Jenner’s cowpox vaccine developed in 1796 to the modern day vaccinia vaccine which helped eradicate smallpox in 1977, have been poorly understood until recently when it was found that neutralizing antibodies (NAbs) are the most critical factor for protection in vaccination [1••,].
As the role of antibodies in protection is supported further, and technologies in engineering antibody Fab and Fc portions are maturing (reviewed in [4•,]), it seems logical to return to the basic question: how do antibodies protect against viruses? Although numerous passive antibody protection studies against different viruses using different animal models have been conducted, we do not seem to have a define answer (reviewed in []). Nevertheless, there are certain generalizations that may hold true for most of the cases: (i) antibodies that neutralize in vitro usually protect better than antibodies that do not neutralize; (ii) non-NAbs may protect but are dependent on Fc-mediated effector functions; and (iii) F(ab’)2 portion of NAbs can also protect although their activity is usually less potent than whole IgG.
In this review, we highlight recent studies relevant to the understanding of virus neutralization and antibody protection. Significant advances have been made to put the interactions of antibody and virus, and the conditions required for neutralization, into a spatial context [7••,]. Furthermore, advances in antibody engineering, especially in the Fc region, have allowed researchers to distinguish the relative importance of antibody-dependent cell-mediated cytotoxicity (ADCC) and complement-dependent cytotoxicity (CDC) in vivo in a monkey model for the first time [9••]. With these new insights, a rational approach to vaccine design has been formulated and will be discussed below.
Mechanisms of antibody-mediated protection against infectious agents
The primary role of antibodies is to prevent infection and to reduce overall viral burden in the host, to an extent that the infection can be contained by ensuing immune responses []. Antibody-mediated protection against infectious agents is based on both direct and indirect functions. The direct function of antibodies, neutralization, refers to the abolition of a pathogen’s infectivity upon antibody binding with no participation of any other components of the innate or adaptive immune system. Neutralization is probably the most powerful function antibodies exert against viruses and it has been demonstrated that removal of the Fc portion did not interfere with the protective capacity of some antibodies despite the decreased half-lives of F(ab’)2 fragments [,].
The Fc-mediated protective functions of antibodies have gained increasing attention with a number of recent studies showing that they can substantially improve the potency of neutralizing antibodies (NAbs) [9••,–]. These functions include activation of the complement cascade, which can result in lysis of the pathogen or infected cells by CDC. Immune complexes (antibody-coated pathogens) can then activate antigen-presenting cells via Fc and complement receptors [], resulting in uptake and processing for antigen presentation to the adaptive immune system. This way, viruses are removed from circulation by phagocytosis [,] and the process of adaptive immune system priming is initiated and accelerated at the same time [,20••]. Other effector functions of antibodies depend on interaction with Fc receptors (FcRs) expressed on immune cells, which is regulated by the combination of antibody isotypes and FcR classes []. ADCC, for instance, describes lysis of antibody-flagged cells by natural killer (NK) cells, which is triggered by signaling of antibodies of the IgG1 and IgG3 bound on the surface of infected cells via the low-affinity FcRγIIIa (CD16a).
The extent to which effector functions contribute to protection appears to be specific for different viruses. In general, in vitro neutralization generally correlates well with protection in vivo. There are only a few exceptions such as Ebola virus where even high titers of NAbs failed to protect monkeys from lethal infection [22•]. Complement has been described to be important for antibody-mediated protection against several viral infections [,]. For HIV, it has been demonstrated that an HIV-1 neutralizing monoclonal antibody (MAb) engineered not to activate complement is as protective as the wild-type antibody. However, when both, complement and FcR binding were abolished, the same antibody showed reduced in vivo protective capacity [9••]. Moreover, complement-mediated virolysis has been described during the acute phase of HIV-1 infection, but it does not seem to have an impact on the overall course of infection []. This clearly indicates that FcR but not complement binding are important to support the in vivo protective capacity of NAbs against HIV-1.
Taken together, these recent data demonstrate that more is yet to be learned on antibody effector functions for in vivo protection of different infectious agents and that the knowledge will greatly benefit the development of therapeutic antibodies optimized towards specific infectious diseases.
Targets for passive and active immunization
Antibody occupancy as a general mechanism for virus neutralization
Considering that neutralization is a shared mechanism for antibody protection against most viruses, it is necessary to understand its molecular basis, to provide useful insight for the development of passive antibodies and vaccines.
The two proposed models concerning the stoichiometry of antibody neutralization of viruses, the single hit and multiple hit models, have been hotly debated in the field although the single hit model has been largely refuted today (reviewed in []). In the single hit model, a single antibody molecule, binding to a critical site on the virus, is sufficient to neutralize a virion. The multiple hit model suggests that multiple antibody molecules are required to neutralize a virion. This model is explained partly by steric hindrance caused by antibody occupancy on viral surface, restricting the viral surface proteins (SUs) of non-enveloped viruses (e.g. VP1, VP2 and VP3 of poliovirus) and enveloped viruses (e.g. gp120 and gp41 of HIV) from interacting with cellular receptors for attachment, or with cofactors essential for post-attachment events of entry (reviewed in [,]). The multiple hit model is gaining further recognition with several recent studies. Yang et al. suggested that to neutralize HIV-1 it would be necessary to occupy every functional envelope (Env) trimer (trimeric complex of gp120 and gp41 heterodimers) with at least one antibody molecule []. Given an average of 8–10 Env trimers are present on each HIV-1 virion [], theoretically it would require ~10 antibody molecules to neutralize an HIV-1 virion. Furthermore, the same group also showed that virus neutralization can be mediated simply by antibody binding without involving any functionally critical site on SUs as proposed in the single hit model []. The authors inserted a foreign FLAG tag into the functionally unimportant V4 region of gp120 of different HIV isolates, and found that an anti-FLAG antibody neutralized the different isolates equally well irrespective of their natural resistance to anti-HIV antibodies, supporting antibody binding/occupancy is the principal determinant for virus neutralization, in agreement with a previous study []. In parallel, Pierson et al. investigated the stoichiometry of antibody neutralization of West Nile virus (WNV) and also found that WNV can only be neutralized by multiple hits, although neutralization was achieved by antibody binding to as few as 30 of the 180 Env proteins present on each virion [7••].
Recent stoichiometric studies have provided additional insights into our understanding of virus neutralization in a spatial context. It was proposed that the extent of antibody coating on virion surface, i.e. the number of antibody molecules, required for neutralization, appeared to be directly proportional to virion size for most viruses []. However, if antibody occupancy on the viral surface is the sole factor dictating virus neutralization, an intriguing question is why more antibody molecules are required to neutralize WNV than HIV-1 (30 versus 10 antibodies/virion) although HIV-1 is significantly larger than WNV (120 versus 50 nm in diameter) [,]? The discrepancy may be explained by a number of factors concerning SU organization on virions (Fig. 1). For WNV and flaviviruses, SUs are packed regularly on the icosahedral virions therefore the level of antibody occupancy required for neutralization is proportional to virus size, as found in many other viruses with regularly packed SUs (e.g. ~38 antibody molecules are required to neutralize papillomavirus which is slightly bigger than a flavivirus, reviewed in []). For HIV-1, SUs (as Env trimeric complexes) scatter sparsely on the viral surface []. Importantly, a single functional HIV Env trimer is sufficient to mediate viral entry. In comparison, for influenza virus, 8–9 Env trimers are required []. In addition, free SUs on a fluid (non-rigid) viral membrane may be able to float away from areas occupied by bound antibodies. Consequently, neutralization of HIV-1 will require occupancy of every Env trimer irrespective of virus size. For more densely packed virions, e.g. WNV, steric hindrance by antibody binding may be extended to neighboring SUs, therefore the level of antibody occupancy required for neutralization may be predictable from virus size. In both viral examples, virus neutralization is mediated by antibody occupancy, but the specific infectivity and organization of SUs vary between the viruses. Therefore, it may be more appropriate to consider antibody occupancy, i.e. the molecular ratio between the total number of available and occupied epitopes, rather than antibodies and virus size.
Factors influencing antibody neutralization of viruses. There are a number of factors in the structure of viruses and antibodies that influence the stoichiometry of neutralization by antibody occupancy. (i) The density of SU packing on the viral surface determines whether steric hindrance due to binding of an antibody to a SU complex can be extended to the neighboring complexes. High antibody occupancy will be required for neutralization of viruses with low SU density. (ii) Spacing between neutralizing epitopes determines if an antibody molecule can bind multivalently. This is dependent on both SU spacing and antibody isotype. If an antibody can bind to more than one SU, low antibody occupancy may be sufficient for neutralization. (iii) Unoccupied SU complexes on enveloped viruses may be able to move laterally to reduce steric hindrance caused by neighboring antibody-bound spikes. Consequently, a higher degree of antibody occupancy is required for neutralization compared to viruses with a rigid SU organization. (iv) Certain epitopes may not be easily accessible to antibodies because they can be occluded intra- and inter-molecularly. Epitopes located in a recessed area or near glycans on SUs, proximal to neighboring SUs or viral membrane, usually require higher antibody concentrations to achieve neutralizing occupancy than well-exposed epitopes. With regard to antibodies, (v) antibody isotype determines the valency, span-width and flexibility of an antibody, and (vi) antibody affinity determines the effectiveness and kinetics of antibody binding. Combining the viral factors outlined above and overall avidity of the antibody/virus interaction, the antibody concentration required to achieve neutralizing occupancy is determined.
Overall, we consider that the basic goal in passive and active immunization is to administer or elicit NAbs of adequate avidity and titer to ensure sufficient occupancy for neutralization. With increasing knowledge of epitopes (see below), SU structures and SU organization, and the number of virus particles required for productive transmission/replication for different viral diseases, it may be possible to derive mathematical models to guide the formulation of passive antibodies for pre-clinical and clinical trials in the future.
The potential advantage of targeting multiple epitopes on viruses
Although antibody occupancy at high affinity and high density should neutralize viruses effectively and provide protection, it is important to note that many dominant epitopes on viral SUs are prone to mutations, or located inside the core of functional SU complexes unavailable for antibody binding (reviewed in [,]). Antibodies to the latter epitopes are presumably elicited by viral debris produced during infection []. For instance, in an analysis of memory B cell antibody responses to SARS coronavirus infection, it was found that ~50% of SU-specific antibodies do not neutralize the virus []. Also when isolated viral SUs are used as an immunogen, they seem to typically induce a large fraction of such non-NAbs (reviewed in []).
Certain RNA viruses and retroviruses, in particular, have developed an array of specialized features in their SUs, e.g. hyperglycosylation on exposed faces and hypermutations at non-essential regions, to escape NAbs (reviewed in []). As a result, these viruses can exist in numerous antigenic or serotypic varieties that cannot be targeted by traditional passive or active immunization. However, there are a few areas on their SUs are refractory to mutation due to functional constraints, e.g. receptor binding site. Therefore, antibodies to epitopes overlapping areas important for SU functions are usually associated with a neutralizing activity against several strains and these antibodies are known as broadly NAbs [4•,36•,]. In an effort to maximize the potency and breadth of antibodies protection against such highly variable viruses, isolate-specific and non-neutralizing antibodies are generally not considered as effective as NAbs targeting conserved epitopes. Currently there is no unified system for the definition of NAb breadth and it is important to clarify that an antibody with broad neutralizing activity does not necessarily mean this NAb can cross-neutralize all viral genotypes or isolates [36•,–]. Nevertheless, the assessment of neutralization breadth of antibodies have been recognized as a key issue in vaccine development in the HIV field and strategies for the analysis of NAb responses induced by immunization have been proposed [].
Important questions are how many conserved neutralizing epitopes exist on different viruses, how these epitopes are arranged on the viral surface, and whether such arrangement of SUs would allow the simultaneous binding of antibodies with complementary specificities thus justifying the cost of developing multiple MAbs? At this juncture, MAb cocktails formulated to target multiple neutralizing epitopes simultaneously are believed to reduce the risk of virus escape and, possibly, enhance neutralization through synergism. Recent studies on MAb cocktails against SARS coronavirus [] and rabies virus (RV) [] have suggested such benefits. More data concerning the efficiency of single MAb formulations and the significance of virus escape are expected from the use of palivizumab, a broadly neutralizing humanized MAb to the F protein of respiratory syncytial virus (RSV), used in prophylaxis against severe RSV disease in high-risk infants and children (reviewed in [4•]). Although escape viruses in cell culture, animal models and humans have been reported for palivizumab, it is unclear if the escape mutants will be pathogenic and selectively amplified in human populations with prolonged use of palivizumab, thus reducing the value of the MAb. It is crucial to follow up the long term effect of palivizumab on circulating RSV to determine if a MAb cocktail is required to target multiple conserved epitopes on RSV and on other infectious agents.
Potential and future of polyclonal and monoclonal antibodies
The current trend in passive antibody development is to replace polyclonal antibodies (PAbs), which are known to be associated with a number of manufacturing and safety issues (see below), with single or multiple MAbs. Another area of interest is to explore whether highly potent MAbs can be used to tackle infections that are not protected by PAbs (e.g. hepatitis C Ig or HCIG [36•,]).
Human PAb products are used in post-exposure prophylaxis against RV, hepatitis A virus and HBV, in prophylaxis against severe illness against RSV, Varicella-Zoster and measles virus in high-risk individuals, and in preventing congenital infection with HBV. PAb preparations are also widely used for organ transplantations to protect organ recipients from emerging cytomegalovirus infections due to immunosuppression, or to protect transplanted livers from recurrent HBV. Another use of PAbs is to reduce severe side effects caused by vaccination against smallpox.
Although these PAb preparations have proven to be effective in preventing and treating viral infections, their human origin leads to batch-to-batch variations and implies a theoretical risk for transfer of unrecognized pathogens. Moreover, shortage of suitable hyperimmune donors, batch contamination by donors who are later found positive for a certain pathogen, and the availability of a limited number of facilities that are licensed to produce such immunoglobulin preparations, lead to frequent albeit temporary shortages of many of these PAb products, and production cannot easily be scaled up in emergency situations. Polyclonal immunoglobulin preparations can also interfere with concomitant or subsequent vaccination against other pathogens. For example, in the case of RSV, the transition from polyclonal RSV-IGIV to monoclonal humanized palivizumab has been favored by its lack of interference with other childhood vaccines [4•]. In other cases, e.g. HBV or RV, passive immunization has not been found to interfere with active immunization and both active and passive immunization is recommended for post-exposure prophylaxis [].
In addition to the advantage of improved safety and specific activity, recombinant MAbs can also be engineered to favor or abolish certain effector functions [,9••,], modified to increase serum half-life, as in case of the third generation therapeutical anti-RSV MAb Numax-YTE [4•], or coupled to (pro-)drugs (gemtuzumab ozogamicin), radioisotopes (ibritumomab tiuxetan chelating 90Y or 111In; 131I tositumomab), or toxins to potentiate their specific activities [].
The main limitation of MAbs, as outlined above, is their mono-specificity that may not cover all naturally circulating antigenic variants of a pathogen, or that may readily select for antibody escape variants. In order to overcome the limitations of single MAb preparations, cocktails of MAbs are currently being pursued for many viral diseases including HBV [], RV [] and SARS [] virus.
Taken together, all these considerations make it likely that most PAb products with a reasonable market will be replaced with recombinant MAbs (or cocktails thereof) in the future. However, for rare applications and those requiring a very broad range of specificities, PAbs may still be favored over blends of monoclonal antibodies.
Knowledge-based vaccine design
For diseases where traditional attempts failed to provide effective vaccines, e.g. HIV and HCV, it will be important to investigate whether a knowledge-based approach can bring new hope to developing vaccines. A considerable amount of scientific knowledge on the requirements for virus neutralization and antibody protection, and on the structures of conserved neutralizing epitopes and SU organization has now been unfolded in recent studies. This should allow one to attempt a rational approach to vaccine development aiming at inducing protective antibody responses. Figure 2 outlines a scheme of knowledge-based vaccine design which is currently under intense research in the HIV research community. This scientific path, also known as “reverse vaccinology or retrovaccinology” [], includes first identifying the specific antibody responses responsible for virus neutralization and protection [36•,], followed by defining the structural determinants in virus neutralization [7,] and by applying the molecular/structural information to designing mimics of the epitopes to focus antibody responses on the broadly neutralizing epitopes in immunization []. It is hoped that this approach can overcome the limitations of traditional vaccine.
Active Vs Passive Immunity
Knowledge-based vaccine design to elicit protective antibodies. To improve vaccine components for the induction of protective antibody responses, one should first define the specificities and magnitude of antibody responses required for protection. One method is to isolate MAbs from subjects who develop protective antibody responses after infection or vaccination, and to identify the MAbs that neutralize the virus in vitro and protect a relevant animal model against virus challenge in passive transfer experiments. Preferably, the MAbs should recognize multiple conserved neutralizing determinants on a given virus. The next step is to define the neutralizing epitopes. X-ray crystallographic and cryo-electron microscopic techniques have recently been applied to dissect neutralizing epitopes structurally and spatially, providing important insights into the conditions required for virus neutralization. The information will provide a template for designing immunogens to favorably present the desired neutralizing epitopes. A major gap requiring further scientific breakthrough is the precise mechanisms of how immunogens are handled and processed in vivo to activate specific B-cells to produce the targeted NAbs. Candidates with the desired antigenic properties will then be screened for immunogenicity in animal models to confirm if the immunogens elicit the desired antibody responses and to protect the animals from virus challenge. Immunogens that produce equivalent or better humoral responses without the side effects associated with traditional vaccines would be a favorable candidate for further clinical evaluation. The molecular models used in the illustration are b12 IgG (Protein Data Bank PDB entry 1HZH) and b12 Fab fragment in complex with gp120 of HIV-1 (PDB entry 2NY7).
Conclusion
Protection by antibodies is a function combining virus neutralization and effector-mediated destruction of virions and infected cells. The multiple dimensions of antiviral activity of antibodies help prevent infection and lower viral burden, thus reducing cell death, extreme immunological reactions and clinical symptoms. We have summarized some of the recent studies that have broadened our thinking about the mechanisms of antibody protection, and that we believe will help the field to refine strategies in improving passive antibodies and vaccine development. The application of multiple advanced techniques including X-ray crystallography and cryo-EM for structural modeling of viral SUs and their packing on viral surface, and the determination of stoichiometry for antibody neutralization of viruses, have not only increased our understanding of the molecular basis of antibody neutralization of viruses but also provided additional rationale in the selection of neutralizing epitopes to target by passive antibodies and the formulation of MAb cocktails. Evidence that different pathogens require different effector functions of antibodies has also created new opportunities in the engineering Fc fragment for passive antibodies, or in the choice of adjuvants and delivery methods in vaccination to favor the induction of a particular antibody isotype, to focus on the most effective effector functions for a particular disease.
Acknowledgement
We thank Pascal Poignard, Dennis Burton, and Eric Rowcliffe for comments, and Christina Corbaci for artwork in Figure 2. Our laboratory is supported by funds from the International AIDS Vaccine Initiative and National Institutes of Health. This is TSRI manuscript number XXX (to be added).
Footnotes
Publisher's Disclaimer: This is a PDF file of an unedited manuscript that has been accepted for publication. As a service to our customers we are providing this early version of the manuscript. The manuscript will undergo copyediting, typesetting, and review of the resulting proof before it is published in its final citable form. Please note that during the production process errors may be discovered which could affect the content, and all legal disclaimers that apply to the journal pertain.
References and recommended reading
Papers of particular interest, published within the period of review, have been highlighted as
• of special interest
•• of outstanding interest
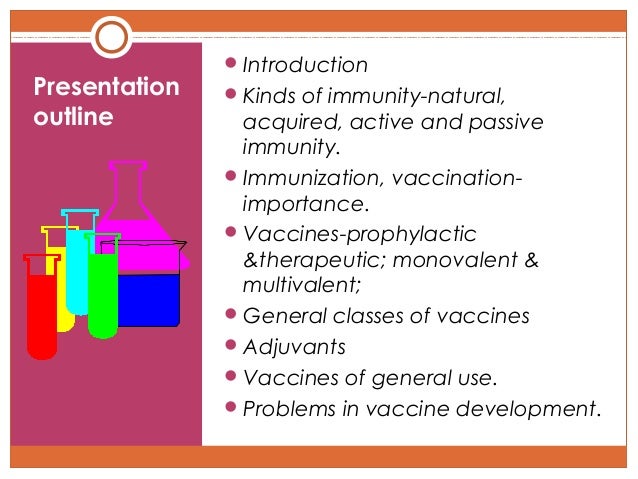
Natural Active And Passive Immunity
